Sample Research Paper
An Introduction to the Origin of Life
by Matti Horne
The beginning of life as we know it has always been shrouded in mystery — and will likely remain shrouded in mystery for the foreseeable future, as far as scientists can tell. There are so many theories regarding our origin story that it’s impossible to discuss them all, but the ones we’ll explore today run the gamut from “we’re all aliens,” or an extraterrestrial origin, to “under the sea,” or at boiling hydrothermal vents on the deep ocean floor. Before we get into the theories, however, we need to understand what our beloved blue marble looked like in its red-hot volcanic infancy, and why the specific conditions on the surface of the early Earth were so perfect for the origin of life as we know it.
Drafts
Writing takes practice and revision. Take a look at Matti’s drafts of her research paper to see how her work changed through her editing process.
Review Matti’s first draft.
Review Matti’s second draft.
Setting the Stage: The Formation of an Early Earth
Around 4.6 billion years ago, approximately 6.5 sextillion tons’ worth of rocks, gas, and dust that had been moving through space got pulled together by gravity. Thermal energy, generated by collisions between the rocks as well as radioactive decay, created a molten, extremely volcanically active proto-Earth. Due to differences in buoyancy, the densest “stuff” in this gooey proto-planet sank, while the lower-density “stuff” rose to the top. This is why Earth has a core made out of iron and nickel, a mantle mostly made up of olivine, and two different types of more silicate-rich crust. The oceanic crust has much more magnesium and iron and is a darker color, while continental crust is lighter in color and primarily consists of a silicate mineral called feldspar. There are different terms for these materials: ultramafic (mantle), mafic (oceanic crust), and felsic (continental crust).
Key Question: Which is denser, oceanic crust or continental crust? How can we tell?
Oceanic crust is much denser than continental crust since it contains a higher percentage of the heavier elements. This is demonstrated by plate tectonics — oceanic crust goes through a process called “subduction,” which means that when two plates collide, the denser one slides underneath, back into the mantle. Since continental crust is the least dense type of crust, it never subducts — like how a pool float is too difficult to pull underwater, but a waterlogged pool noodle will slide under without much resistance.
As the surface of the early Earth slowly began to cool, the ancient atmospheres and oceans were able to collect and condense. Their compositions were drastically different from what we know today, with compounds provided entirely through a process called “degassing.” Molecules such as water and carbon dioxide that were “locked” in minerals and bubbles in the early mantle and crustal rocks managed to escape and exist as gases or liquids through volcanic eruptions.
Key Question: Is there any more water or air “locked” in the mantle? How can we tell?
There is still a huge amount of water “locked” in the mantle — anywhere from 3 to 90 times that stored in our present-day oceans! Although this sounds impossible, this “water” actually occurs as “point defects” — singular hydrogen atoms trapped in various minerals’ crystal structures (also called “lattices”), like many millions of tiny mutations in the minerals’ DNA. We know this by studying the chemical compositions of the lava and gas expelled in modern-day volcanic eruptions.
Atmospheric Composition
As you can see, the early atmosphere primarily consisted of carbon dioxide instead of nitrogen, and this likely played a large role in the ancient ocean’s composition, which we know to be much more acidic than it is today. Its low pH was likely due to large amounts of dissolved CO2 in the early water, which essentially ‘hangs out’ (fun term!) as carbonic acid. Some scientists have even speculated that the ocean was “carbonated” by our modern standards and had a fizzy quality. Its acidity likely made it more efficient at weathering and eroding rocks that it came into contact with, whether those were on the early seafloor or at the edges of the early continents.
Key Question: What’s different about the ancient atmospheric composition, compared to our current-day atmosphere?
There was no free oxygen in Earth’s ancient atmosphere! Any oxygen molecules that escaped from volcanic eruptions, degassing, or early photosynthesis likely became dissolved in the oceans, where it reacted with iron atoms. The newly “rusted” molecules were then precipitated onto the seafloor, creating bright red, striped deposits called Banded Iron Formations, or BIFs. All of the free oxygen in the atmosphere that makes life as we know it possible came from photosynthetic reactions. The first life-forms to integrate photosynthesis into their DNA were called cyanobacteria!
Key Question: Would the ancient atmosphere have different properties?
An atmosphere mostly consisting of CO2 would have been much denser and “heavier” than what we’re used to now. This drastic difference in density could have increased the pressure at the Earth’s surface to as high as 100 atm (our standard sea-level pressure today is 1 atm), which could allow the oceans to reach temperatures up to 100° C without boiling. This high CO2 content in the ancient atmosphere likely also translated to the high CO2 content in the ancient oceans.
The following table shows a comparison between the ancient atmospheric composition, which was formed from the gas emitted through volcanic eruptions, and the present-day atmospheric composition, which has been altered drastically by the presence of life.
Ancient | Present Day |
Primarily carbon dioxide | Nitrogen (78%) |
Hydrogen sulfide | Oxygen (21%) |
Water vapor | Argon (0.9%) |
Sulfur dioxide | Water vapor (0 – 4%) |
Volcanic gases (“VOG”) | Trace gases (includes carbon dioxide) (0.1%) |
The Next Step: The Basic Chemistry of Early Life
Scientists have pretty much ruled out the possibility that fully-formed life either a) spontaneously popped into existence or b) traveled here on a meteorite, crash-landed on early Earth, and promptly set up camp. So how did life as we know it come to be?
The commonly accepted ‘precursors to life’ are called enantiomers: molecules made up of different arrangements of organic compounds — carbon, hydrogen, nitrogen, oxygen, phosphorus, and sulfur, colloquially known as CHNOPS. Enantiomers come in pairs, and the two paired molecules are mirror images of one another. One group faces left, and thus is referred to as “left-handed” or “L-enantiomers,” while the other group faces right, and is called “right-handed” or “R=D-enantiomers.” It turns out that the sunlight we receive on Earth’s surface is polarized in such a way that D-enantiomers break down much more quickly than L-enantiomers. On top of that, cells can only consume one group of enantiomers (either L- or D-). Since L-enantiomers are more stable on Earth, it made sense for the first cells — and, consequently, every organism after that first cyanobacteria — to use L-enantiomers for their building blocks, and (mostly) L-enantiomers for their food. This means that all of the forms of life that we have studied so far are made up almost entirely of L-enantiomers — and so scientists have concluded that a preexisting overabundance of L-enantiomers would have been more conducive to the formation of life.
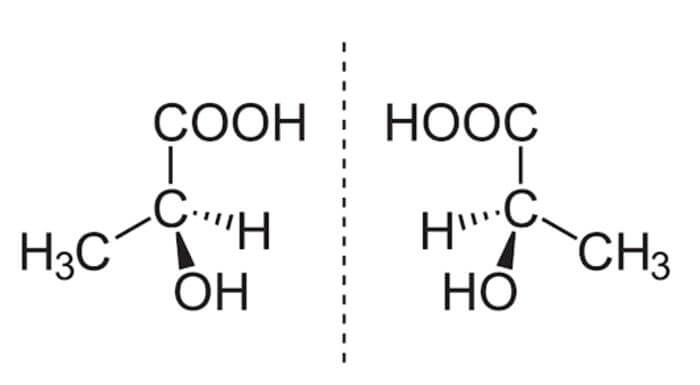
An example of a pair of enantiomers — note how they are mirror images of each other. On the left is a left-handed version of a compound called lactic acid; on the right is the same molecule arranged in a right-handed fashion.
Open versus Closed Chemical Systems
There were (and still are) many different types of chemical reactions occurring between the ocean, the rock, and the atmosphere. We can classify these various reactions as belonging to “open” or “closed” systems. “Open” systems include interactions between all existent phases; in this case, those are the ocean, the atmosphere, and the rocks. Locations where such interactions could have occurred include:
- early coastlines, cliffs, or shallow seas where wave action oxygenates the water and breaks up rocks
- weathering and erosion due to early precipitation; sediments could interact with the atmosphere and ocean during the runoff process
The “closed” systems, on the other hand, accounted for reactions between only two of the three available phases — for example:
- deep-sea reactions (e.g. at hydrothermal vents) between ocean and rock
- gas exchange between the atmosphere and the ocean.
It is also important to note that, since different reactions occurred in open and closed systems, the secondary minerals and fluids produced likely varied between systems. This wide range of secondary minerals and fluids created a chemical disequilibrium — this lack of balance facilitated many chemical reactions that could have created ideal conditions for the origin of life.
Key Question: Can a system ever be completely “closed”? Why, or why not?
No system is ever completely “closed.” For example, nutrients from hydrothermal vents eventually make their way to the surface through upwelling, in the same way that gaseous or acidic CO2 dissolved into the top level of the ocean eventually makes its way to the seafloor through the mixing of ocean currents. Although these processes can take thousands of years, they can still influence chemical reactions occurring in a “closed” system.
Current Theories on the Origin of Life
First of all, let’s consider hydrothermal vents. Formed on the ocean floor, hydrothermal vents are connected to underwater volcanism and usually occur near or on top of mid-ocean ridges. These vents emit heated, chemically modified seawater that has high concentrations of CO2, sulfides, and metals from being cycled through the oceanic crust below. Evidence of life, from fossils dating back to 90 million years ago up to current-day living organisms, has been found at hydrothermal vents — extremophiles and anaerobic bacteria thrive in the chemically-saturated, high-energy environments. Since life has existed at these vents for so long, and since hydrothermal activity has been ongoing since there was a liquid ocean on Earth – about 4.2 billion years ago, it seems reasonable that life could have originated at these sites.
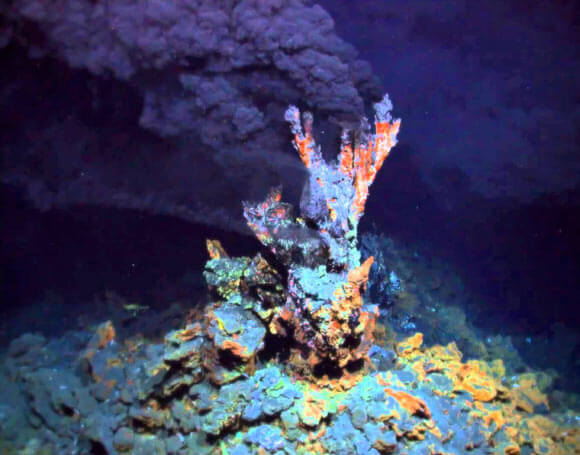
A hydrothermal vent at the bottom of the ocean. Note the bright colors deposited on the sides of the vent — likely extremophile bacteria as well as sulfide minerals — and the black “smoke” of heated, nutrient-rich seawater emitting from the vent. Image credit: Oregon State University / CC BY-SA 2.0.
A second hypothesis focuses primarily on clay minerals and the chemical reactions they facilitate. Produced from the weathering of ultramafic and mafic rocks, clay minerals could have formed around mid-ocean ridges, early underwater volcanoes, hydrothermal vents, or even along early shorelines. Since clay minerals have such a high surface area, they tend to be able to concentrate various organic molecules and provide a calmer, more sheltered environment for them to bump into each other and react, possibly forming life! Additionally, the minute variations in the arrangement of atoms and molecules in a mineral’s molecular structure (also called a “lattice”) results in different charges on the different crystal faces — and this can actually attract different molecules! This process also applies to organic molecules, such as enantiomers — the commonly accepted precursor to life. In an experiment with calcite crystals, for example, one specific face attracted L-enantiomers (which, as we discussed, are preferred by life as we know it). Although calcite is not a clay mineral, this “face preference” phenomenon is also present in many other varieties of minerals and crystals. In combination with the sheltered “molecule nurseries” provided by clay minerals, face preference could have been conducive to speeding up chemical reactions that eventually led to life!
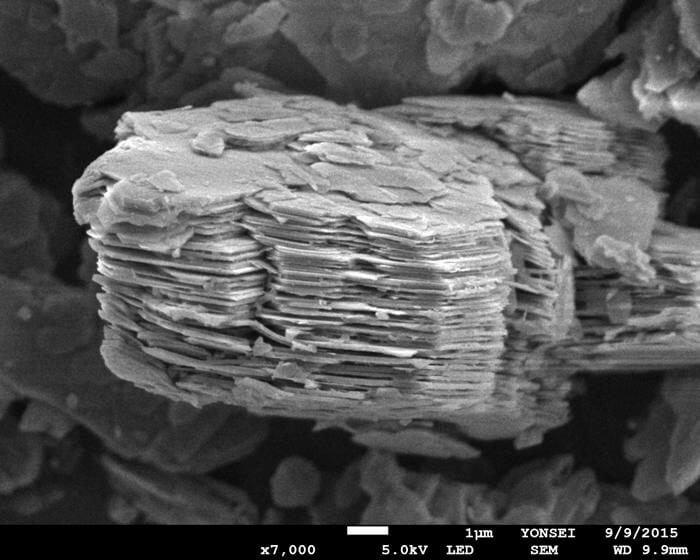
Note the many microscopic layers in this photograph of kaolinite, a clay mineral. Photo credit: Yonsei University, Yongjae Lee.
The third theory that we’ll explore today is most definitely the flashiest: meteorites. This “extraterrestrial life” theory certainly has sci-fi appeal, but does it hold scientific weight? Although it is highly unlikely that fully-formed life (e.g., single-cell or multicellular life-forms) traveled here on a meteorite, in a recent study, bacterial spores were shown to have the abilities to withstand the immense temperatures and pressures resulting from a meteorite impact. Additionally, there have been several studies of the amino acids found within the mineral composition of carbonaceous chondrites. The chemistry of the Murchinson meteorite (landed in 1969 in Australia), for example, has been extensively studied and characterized. Not only were the amino acids found within the Murchinson sample indigenous to the meteorite, but the molecules were also dominated by L-enantiomers — pointing to the possibility that the origin of life was, indeed, interstellar.
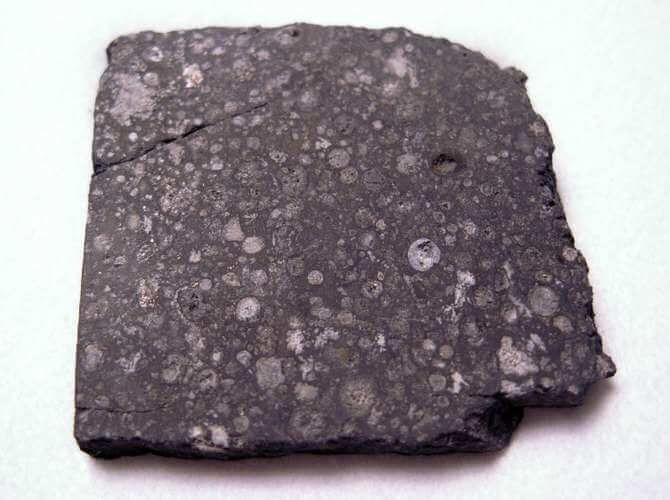
A slice of the Allende meteorite, also a carbonaceous chondrite. The circular patches are called “chondrules.” Meteorites without these spheres are called “achondrites,” meaning “without” or “lacking” chondrites. Photo credit: Jon Taylor (CC BY-SA 2.0).
In Conclusion
At the moment, there is no one “correct” explanation for the origin of life — mostly because we have no geologic, chemical, or biological record from the early Earth due to its violent and transient nature. However, there is also the possibility that life happened to evolve multiple times in multiple different localities — it was almost certainly not a single coincidence that led to where we are today. Robert Hazen claims that “chance” versus “necessity” is a false dichotomy — a chemical reaction that is improbable to occur on a laboratory scale over a day, a few months, or a year, is essentially inevitable on a planetary scale over millions of years. Additionally, it might not have been only clay minerals or only hydrothermal vents that provided a catalyst for the first life-forms — it may have happened due to the coincidental presence of a clay mineral “molecule nursery” near a hydrothermal vent, which provided heat, nutrients, and a mix of catalysts to further the reaction. Any number of these factors could have combined to produce the perfect storm — but with our current technologies, we can only guess.
Sources
Cronin, J.R., and S. Pizzarello. “Amino Acids in Meteorites.” Advances in Space Research, Pergamon, 23 Oct. 2002, www.sciencedirect.com/science/article/pii/0273117783900364.
Engel, M. H., and S. A. Macko. “Isotopic Evidence for Extraterrestrial Non- Racemic Amino Acids in the Murchison Meteorite.” Nature News, Nature Publishing Group, www.nature.com/articles/38460.
Hazen, Robert M. “Chance, Necessity, and the Origins of Life.” Lecture, Woodford-Eckis, Claremont, 28 February 2020.
Hazen, Robert M. “THE STORY OF EARTH: How Life and Rocks have Co-Evolved.” Lecture, Woodford-Eckis, Claremont, 29 February 2020.
Horneck, G., et al. “Bacterial Spores Survive Simulated Meteorite Impact.” Icarus, Academic Press, 25 May 2002, www.sciencedirect.com/science/article/pii/S0019103500965436.
Martin, William, et al. “Hydrothermal Vents and the Origin of Life.” Nature News, Nature Publishing Group, 29 Sept. 2008, www.nature.com/articles/nrmicro1991.
Morse, J.W., Mackenzie, F.T. “Hadean Ocean Carbonate Geochemistry.” Aquatic Geochemistry 4, 301–319 (1998). https://doi.org/10.1023/A:1009632230875
Meunier, A., Petit, S., Cockell, C.S. et al. “The Fe-Rich Clay Microsystems in Basalt-Komatiite Lavas: Importance of Fe-Smectites for Pre-Biotic Molecule Catalysis During the Hadean Eon.” Orig Life Evol Biosph 40, 253–272 (2010). https://doi.org/10.1007/s11084-010-9205-2
Pizzarello, Sandra. “The Chemistry of Life’s Origin: A Carbonaceous Meteorite Perspective.” Accounts of Chemical Research, vol. 39, no. 4, 2006, pp. 231–237., doi:10.1021/ar050049f. (https://pubs.acs.org/doi/pdf/10.1021/ar050049f.)
Sahai, Nita, et al. “The Transition from Geochemistry to Biogeochemistry.” Elements, Volume 12, Number 6, pp. 389–394 (2016).
Schoonen, Martin, Smirnov, Alexander. “Staging Life in an Early Warm ‘Seltzer’ Ocean.” Elements, Volume 12, Number 6, pp. 395-400 (2016).
Wellford, Sebastian. “Biological Homochirality: One of Life’s Greatest Mysteries.” Medium, Cell Your Soul, 13 July 2016, medium.com/a-spoonful-of-sugar/biological-homochirality-one-of-lifes-greatest-mysteries-2031f4700c4b.
0 Comments